BIOCHEMISTRY TOPICS
Lysozyme
Lysozyme catalyzes the hydrolysis of glycosidic bonds in bacterial cell wall peptidoglycan. The role of binding energy in catalysis by lysozyme. Distortion of substrate into a higher energy conformation that resembles the transition state. General acid and general base catalysis in lysozyme, and importance of a perturbed pKa. Further features of the lysozyme mechanism.
Lysozyme, a 14.6-kD globular protein discovered by Alexander Fleming in 1922, provides a useful example illustrating the role and importance of binding energy in enzymatic catalysis. The bactericidal effect observed by Fleming is due to its activity as an enzyme [EC 3.2.1.17] that hydrolyzes glycosidic bonds between the monosaccharide units of bacterial peptidoglycan cell wall. Lysozyme occurs in orthologous forms in many different organisms. One of the best studied forms occurs in relatively high amounts in hen egg white (often abbreviated HEWL). Another quite well studied form is T4 lysozyme, from T4 bacteriophage. It performs the same catalytic function as avian and other lysozymes from higher organisms, exhibits similar structural and binding properties, but has no detectable sequence similarity. There is just enough structural similarity between HEWL and T4 lysozyme to suggest that both proteins are evolutionarily related through a distant common ancestor. Lysozyme functions as an antibacterial agent, and in some cases plays a role in digestion.
Lysozyme also provides a foundational example of how the structural studies of enzymes using X-ray crystallography yield indispensable information about the mechanistic features of enzymatic activity. In fact, lysozyme was the first enzyme whose structure was determined crystallographically, this feat having been accomplished in the laboratory of D.C. Phillips by 1965. Lysozyme was by no means an ideal target for enzymological investigations because of the complexity of its substrate. Nonetheless, the structural information obtained by Phillips and coworkers was sufficient to allow them to propose a plausible model for the catalytic mechanism. Research since that time has largely validated their model, although new complexities and remaining unanswered questions color our current perspectives on this archetypical example of structure-function studies of enzymes.
The structure of lysozyme shows a roughly ellipsoid shape, with axes of about 42 and 30 Å, largely composed of α helix, with 15% β sheet. The helices are stacked on either side of a single, twisted antiparallel β sheet, with the latter forming an important part of a cleft that runs across an entire face of the molecule. This cleft constitutes the binding site for the substrate glycan chain.
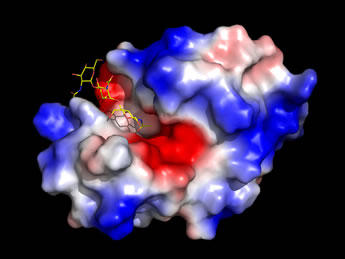
Lysozyme: Substrate & specificity
The cell walls of bacteria are composed of a highly crosslinked peptidoglycan that is composed of long polysaccharide (glycan) chains that are linked by pentaglycine bridges and tetrapeptides with D-amino acid residues. The repeating unit of the polysaccharide chains is an N-acetylglucosamine, N-acetylmuramic acid disaccharide. In the crosslinks, the lactyl side chain of N-acetylmuramic acid (NAM) forms an amide linkage with a tetrapeptide. The O-glycosidic linkages between N-acetylglucosamine (NAG) and NAM are all of the β(1→4) type that link the C1 and C4 atoms of the sugars. Lysozyme specifically hydrolyzes the glycosidic bond between C1 of NAM and O4-C4 of NAG, although it shows hydrolytic activity toward other similar substrates.
The key active site residues are Glu35 and Asp52, while Trp62, Trp63, Asp101, and Trp108 are involved in substrate binding. These residues line the cleft and yet are well separated in the sequence.
Lysozyme: Catalytic mechanism
As lysozyme was the first enzyme for which a structure was obtained by X-ray crystallography, it also provided the first opportunity to use the structure of an enzyme to guide proposal of a mechanism for its catalytic function.
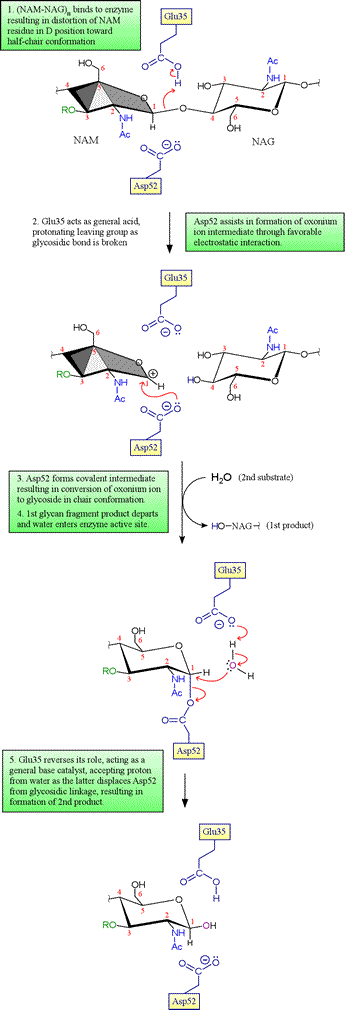
Initially, Phillips and coworkers had only the structure of the free enzyme, and thus no direct experimental evidence for the structure of the enzyme in complex with its substrate was available. However, modeling of the substrate into the active site cleft indicated that six monosaccharide residues of the NAG-NAM glycan copolymer (designated by the letters A-F) could be well accommodated with the following caveats: occurrence of steric clashes dictated that (i) residues A, C and E must be NAG, as the bulky lactyl group attached to C3 of NAM created steric clashes for residues C and E, and (ii) the residue corresponding to D could not fit in its most stable chair conformation, but could be accommodated if distorted toward a half-chair conformation. This latter observation becomes quite important in a proposed mechanism involving an oxonium ion intermediate, since the distorted half-chair conformation resembles the structure of such an oxonium intermediate.
The key residues Asp52 and Glu35 play two distinct roles in the catalytic mechanism of lysozyme. Asp52 is in a relatively polar environment, and has a normal pKa. Its negative charge stabilizes a developing positive charge as the reaction proceeds through the oxonium ion intermediate. However, the γ-carboxyl group of Glu35 is in a more nonpolar environment, and has an anomalously high pKa (its pKa is thought to be about 6.5), keeping it more in the protonated form. The unionized form of Glu35 donates a hydrogen ion to the oxygen of the glycosidic linkage, assisting the breaking of the bond between sugar residues. Glu35 would not be so effective in this role if its pKa was normal.
Evidence for the covalent intermediate. In the original Phillips mechanism, water adds directly to the oxonium ion intermediate, with retention of the β anomer if water attacks the same side of the ring from which the 4-oxygen of the first product glycan departed (on the Glu35 side of the oxonium, as shown in the second panel above; this would be denoted as the si face of trigonal planar sp2-hybridized oxonium carbon). This mechanism was accepted for many years, despite the fact that such an oxonium ion would be expected to be quite unstable in the presence of any nucleophilic species, and in particular much less stable than an acyl glycoside (as in the Asp52-linked covalent intermediate). Direct evidence for either an oxonium ion intermediate or an acyl glycoside intermediate was lacking despite sustained efforts to distinguish between the two alternatives. In 1993, studies of a mutant version of T4 lysozyme carried out by Brian Matthews and coworkers revealed a covalent adduct between substrate and enzyme. Then in 2001, Withers and associates were able to employ the E35Q mutant of HEWL along with a cleverly-designed modified substrate to trap the enzyme with substrate covalently attached to Asp52 (you can view this structure by downloading the 1H6M pdb file and copying and running the lzm_1H6M PyMOL script linked to below). In view of these results, the mechanism of lysozyme now enjoying the greatest acceptance includes the covalent intermediate as depicted above, and the oxonium ion is viewed generally as a very unstable intermediate species at best, essentially equivalent to a transition state leading to and from the acyl glucoside intermediate.
PyMOL scripts for lysozyme |
![]() |
![]() |
||||