Lecture 26. Oxidative decarboxylation
Tuesday 30 April 2019
Carboxylation and decarboxylation reactions in biochemistry: a perspective. The fate of pyruvate, a key metabolic intermediate. Pyruvate carboxylase is a biotin-dependent carboxylase that serves gluconeogenesis and/or anaplerotic production of oxaloacetate. Oxidative and non-oxidative decarboxylations. Conversion of pyruvate to acetyl CoA pyruvate dehydrogenase complex {PDH complex}. A key coenzyme, (TPP) cofactor: TPP chemistry, structure, and role in the PDH mechanism. PDH complex: Structure and function. Citric acid cycle overview.
Reading: Lehninger - Ch.14, pp.553-558; Ch.16, pp.619-624.
Bridging glycolysis and the citric acid cycle
Pyruvate, the end product of glycolysis under aerobic conditions, is a metabolic branch point. As a preliminary to following the central path of aerobic metabolism from glycolysis to the citric acid cycle, we put pyruvate in perspective by considering its various possible fates. We also consider the broader context of common carboxylation and decarboxylation reactions in biochemistry.
The most important fate of pyruvate - at least for our present purposes - is its oxidative decarboxylation to acetyl CoA, This reaction is catalyzed by a very large assembly of enzyme subunits called the pyruvate dehydrogenase complex (PDH complex, or often simply "PDH"). This large supramolecular assembly contains multiple copies of three different types of subunit. These subunits catalyze different steps of the overall reaction. Central to the operation of the PDH complex is a key catalytic cofactor, thiamine pyrophosphate (TPP). We will examine closely the chemistry of this extraordinary and important cofactor.
Carboxylation and decarboxylation reactions
Let us define a carboxylation reaction as the addition of a CO2 unit to a substrate molecule, and decarboxylation as loss of CO2. Decarboxylation reaction reactions are typically quite thermodynamically favorable due to the entropic contribution of cleaving a single molecule into two, one of which is a gas. Conversely, we can expect carboxylation reactions to be energy-requiring, and we should not be surprised to learn ATP hydrolysis is coupled to carboxylation. The most prominent carboxylation reactions in biochemistry are catalyzed by biotin-dependent carboxylases and RuBisCO.
The biochemistry of thiamine
Decarboxylations in metabolism can be either non-oxidative or oxidative. In contrast to the relatively facile decarboxylation of β-keto acids, the decarboxylation of α-keto acids presents a mechanistic challenge. Thiamine pyrophosphate (TPP) provides the biochemical and enzymological answer.
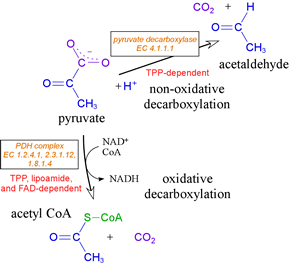
TPP is the key catalytic cofactor used by enzymes catalyzing non-oxidative and oxidative decarboxylations of α-keto acids. Pyruvate, for example, undergoes both types of decarboxylation, both involving TPP. In fermentative organisms, pyruvate is non-oxidatively decarboxylated by the TPP-dependent enzyme pyruvate decarboxylase. As part of the PDH complex, TPP assists in oxidative decarboxylation of pyruvate. TPP is a true catalytic cofactor. In a mechanistic feature common to all of its reactions, TPP is a carrier of activated aldehyde moeities.
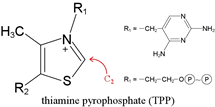
A hydrogen attached to the C2 carbon of the thiazole ring of TPP shows an unusually low pKa.
Thiamine deficiency underlies the disorder beriberi.
The pyruvate dehydrogenase (PDH) complex
Oxidative metabolism entails the further catabolism of pyruvate. The pyruvate dehydrogenase, or PDH complex carries out the oxidative decarboxylation process that generates acetyl CoA from pyruvate. The PDH complex serves as the link between glycolysis and the citric acid cycle, and is required for oxidative metabolism. The activity of PDH involves three distinct enzymes, four activities, and five different cofactors (see VVP4e, pp.555-561).
Steps of the PDH complex:
(1) decarboxylation (E1, formation of hydroxyethyl-TPP)
(2) oxidation (transfer of acetyl group to lipoamide)
(3) transfer of acetyl group from acetyllipoamide to CoA)
(4) oxidation of dihydrolipoamide to lipoamide (E3, FAD, NAD+)
Acetyl CoA, which is fed into the citric acid cycle This conversion, an oxidative decarboxylation of pyruvate yielding a thioester product, is carried out by a complex and fascinating multienzyme complex known as the pyruvate dehydrogenase (PDH) complex (also sometimes abbreviated as PDC).
Multiple copies of three different enzymes - E1, E2, and E3 - compose a supramolecular structure that coordinates a four step process converting the α-keto acid pyruvate to the thioester (with coenzyme A) of acetate, as well as electron transfer (redox) reactions that yield NADH. Five cofactors participate in the reactions of the complex. E1 is pyruvate dehydrogenase [EC 1.2.4.1], which uses thiamine pyrophosphate (TPP) as a cofactor to decarboxylate pyruvate and transfer the remaining hydroxyethyl fragment to the lipoamide cofactor attached to E2. This results in the formation of acetyllipoamide, equivalent to reduction of lipoamide (and oxidation of the hydroxyethyl fragment), as becomes clear upon subsequent transfer of the acetyl residue to coenzyme A, catalyzed by E2, a acetyltransferase [EC 2.3.1.12] and E3, which regenerates lipoamide from dihydrolipoamide, is dihydrolipoyl dehydrogenase [EC 1.8.1.4].
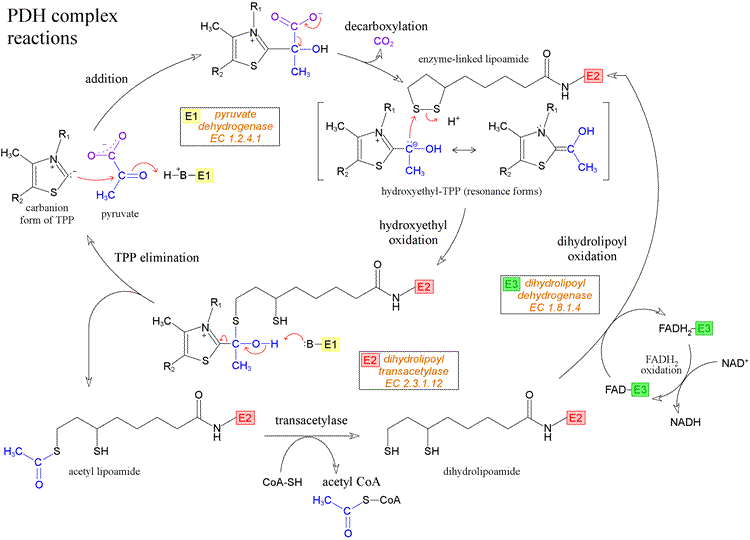
The diagram above shows the reactions of the PDH complex. Starting at the left-hand side, the thiazolium form of the TPP cofactor, which is a carbanion resulting from loss of H+ from the unusually acidic C2 of the thiazole ring, attacks the carbonyl carbon of pyruvate, forming the addition compound shown at the top of the figure. This addition compound can readily undergo decarboxylation (loss of carbon dioxide), with the product hydroxyethyl-TPP stabilized by resonance. The next step is the transfer of the hydroxyethyl moiety from TPP to the oxidized form of the lipoamide cofactor. The hydroxyethyl group is electron-rich, and in its reaction with lipoamide it is in effect oxidized to the carboxyl level of oxidation, while lipoamide is reduced. This reaction can be dissected into two steps, where in the first step the electron-rich carbon atom of the hydroxyethyl group attached to TPP attacks - as a strong nucleophile - one of the relatively electron-deficient sulfur atoms of the intramolecular disulfide of oxidized lipoamide. This results in the intermediate shown at lower left in the figure, which has the form of a hemithioketal. In the next step, as TPP departs as a leaving group, taking electrons from the bond to the hydroxyethyl group with it, the hydroxyethyl recruits the electrons from the O-H bond, assisted by a conveniently located enzyme-derived base to accept the resulting hydrogen ion. The result of these two steps is the production of the thioester acetyl lipoamide and regeneration of the TPP cofactor. All of these reactions are catalyzed by the E1, or pyruvate dehydrogenase, component of the PDH complex.
The rest of the chemistry of the PDH complex is shown at the bottom of the figure. The acetyl group is transferred from reduced lipoamide to coenzyme A (CoA) by the activity of the E2, or dihydrolipoyl transacetylase, component of the complex. This is an isoenergetic conversion of one thioester to another. The free dihydrolipoamide (reduced form of lipoamide) must be re-oxidized, and this is accomplished by the activity of E3, or dihydrolipoyl dehydrogenase, component of PDH complex. Note that the cofactor of E3 is a tightly-bound flavin adenine dinucleotide (FAD) molecule. The electrons from dihydrolipoamide are transferred, via FAD, to NAD+, forming NADH. This is noteworthy since in the usual order of reduction potentials, the reduction of FAD by NADH would be the energetically favorable process. Apparently, specific protein environments are able to perturb reduction potentials of redox groups, just as they are able to perturb pKa's of ionizable groups.
A very similar series of reactions is carried out as part of the citric acid cycle by the α-ketoglutarate dehydrogenase multienzyme complex, which is also closely related to the PDH complex in composition and structure, using the same E3 component, and an E1 that acts on α-ketoglutarate as a substrate in place of pyruvate. In this case, E2 is a succinyltransferase, and succinyl CoA is the product thioester.
Structure of the PDH complex
The PDH complex is a very large structure containing multiple copies of the E1, E2, and E3 components, which are themselves multimers of individual polypeptide chains. There are two different morphologies of PDH complexes: an octahedral complex found in gram-negative bacteria (e.g. E. coli), and a larger icosahedral complex found in eukaryotes and gram-positive bacteria. The complex from E. coli has a molecular weight of 4.6 MD (megadaltons) Additional components are present in the eukaryotic complexes.
The complex from E. coli is built up from a cubic core of eight E2 (transacetylase) components. Each E2 is a trimer, α3, made up of three subunits, so that the E2 core contains 24 total separate, but identical, polypeptide chains. Two dihydrolipoyl dehydrogenase (E3) components are associated with each face of the cube defined by the E2 core, for a total of 12 E3's. Each E3 is actually a dimer, so there are 24 subunits contributed by the 12 E3 components. Two E1 (pyruvate dehydrogenase) components are then placed along each of the 12 edges of cube, for 24 E1 components. Each E1 from E. coli is actually a dimer, so there are 48 separate polypeptide chains contributed by the E1 components. The overall architecture has an octahedral (cubic) symmetry, as noted. The architecture of the PDH complex is strikingly evident in electron micrographs, also underscoring how large this complex is.
A noteworthy feature of the structure-function relationships in the PDH complex is that the long (~14 Å) lipoyl-lysine arm allows functional cooperation between the subunits of the complex. This permits the complex chemistry of the PDH reactions shown above to occur in different active sites without the release of intermediates, as the lipoyl-lysine arm is thought to literally swing from one active site to another.