BIOCHEMISTRY TOPICS
Biological oxidation-reduction reactions
Review of definitions and conventions from general chemistry. Assigning oxidation states to carbon atoms in Lewis structures. Oxidoreductase enzymes and biological redox cosubstrates.
Oxidation-reduction (or "redox") reactions are a very large class of chemical reactions in which both oxidation and reduction necessarily occur.
An oxidation is defined as loss of electrons in the course of a chemical reaction. If a species gains electrons, it is undergoing a reduction. Since electrons are "conserved" in a chemical reaction (they are not created or destroyed),one chemical species' loss is another's gain. Thus, a reduction cannot occur with a corresponding oxidation, and vice-versa - a kind of yin-yang principle. The term "redox" also nicely encapsulates how inextricably tied together oxidation and reduction are in reality. Other terminology used in discussing redox chemistry: A chemical species that gets reduced is acting as an oxidizing agent, or oxidant, while the species undergoing oxidation is acting as the reducing agent, or reductant.
Oxidation state (or oxidation number) is a bookkeeping device employed by chemists to help them classify and understand chemical reactions. The simplest way to interpret oxidation number is to think of it as the number of electrons lost or gained by an atom (compared to its neutral, uncombined form) when it reacts to form ions or molecules. Consider first the case of ions. For monatomic ions, such as Na+ or Cl−, the oxidation number is the same as the charge, +1 and −1, for the sodium cation and chloride anion, respectively. In molecules and polyatomic ions, oxidation states for atoms are calculated by comparing the number of valence electrons in the neutral atom with a count of the surrounding bonding and nonbonding electrons in the Lewis structure. In this respect, it is similar to determining formal charge of an atom in a Lewis structure. A general rule in determining an oxidation number of an atom in a complete Lewis structure, is any differences in electronegativity between covalently bonded atoms is treated as if the bond is actually ionic. That means both electrons are counted as belonging to the more electronegative atom. Note how this differs from formal charge: In computing formal charge, we treat electrons in covalent bonds as equally shared, despite differences in electronegativity. But like formal charge, the sum of the oxidation numbers for each atom in a formula or Lewis structure for a molecular or ionic species must sum to the net charge of that formula or Lewis structure (zero for a molecule). We will soon see that assignment of oxidation numbers and following how they change in a chemical reaction allows us to recognize redox reactions and determine the stoichiometry of the electron transfer occurring.
It is easy to recognize any reaction featuring an uncombined, neutral element as a redox reaction. Some examples are
CH4(g) + 2 O2(g) → CO2(g) + 2 H2O(l)
Fe(s) + O2(g) → Fe2O3(s)
2 Na(s) + Cl2(g) → 2 NaCl (s)
which represent combustion of methane, rusting of iron, and the formation of salt. In the first, the elemental form of oxygen reacts, with the oxygen ends up in carbon dioxide and water. In the second, two uncombined elements, a metal and nonmetal react to form an ionic compound. In the third example, an ionic compound is again formed from combination of elemental forms (metal and nonmetal), but oxygen is not involved, illustrating that chemists have generalized the original concept of oxidation. What if there are no uncombined elements in a chemical reaction? Is it still possible to have a redox reaction? The answer is yes, and the following reactions provide examples:
8 H+(aq) + 2 NO3−(aq) + 3 Sn2+(aq) → 4 H2O(l) + 2 NO(g) + 3 Sn4+(aq)
16 H+(aq) + 3 C2H5OH(aq) + 2 Cr2O72−(aq) →
11 H2O(l) + 3 CH3CO2H(aq) + 4 Cr3+(aq)
The first reaction clearly shows the tin(II) ion being oxidized to the tin(IV) ion. The accompanying reduction is less easily picked out. The second reaction, which is the basis for the Breathalyzer™ test, might be suspected of being a redox reaction by noting that chromium (Cr) appears in two different ionic forms, and that ethanol (C2H5OH) is being converted to a compound (CH3CO2H, acetic acid) containing more oxygen and less hydrogen in combination with a fixed number (two) of carbon atoms.
In order to identify in all cases whether a given reaction is an oxidation-reduction reaction, and to also determine the electron stoichiometry of a redox reaction, we use a set of bookkeeping rules to assign oxidation states to each individual atom in the molecules and ions involved in a given chemical reaction. We look for changes in oxidation states, and when oxidation states change in a reaction we can say the reaction is a redox reaction. If the oxidation state of an element decreases in a reaction, it is undergoing reduction. In cases where oxidation state of an element increases, that element is being oxidized.
Rules for assigning oxidation states
The rules for assigning oxidation states are fairly simple. The following formulation is based on that given by Tro (Ref. 3, below).
- The oxidation state of an atom in an elemental form is zero.
- The oxidation state of an atom in monatomic ionic form is equal to the charge on the ion.
- The sum of all oxidation states in a molecule, polyatomic ion, or formula unit is always equal to the net charge.
-
A hierarchical series of oxidation states for elements, based fundamentally on their valences and
the
electronegativity scale,
is used to assign oxidation states for individual elements as follows:
- Group I elements (Li, Na, K,...) always have a +1 oxidation state in their compounds.
- Group II elements (Be, Mg, Ca,...) always have a +2 oxidation state in their compounds.
- Fluorine always takes on the oxidation state of −1 in its compounds.
- If not dictated otherwise by the above, hydrogen will have an oxidation state of +1 in its compounds.
- Unless dictated otherwise by the above rules, oxygen will have an oxidation state of −2 in its compounds.
- If not determined already by the rules, other elements will have the following oxidation states (in order of precedence): halogens besides fluorine (Cl, Br, I), −1; sulfur (S) −2, nitrogen (N) −3.
- Assignment of oxidation state for any remaining elements not yet assigned should in most cases be possible by application of rule 3.
Identifying oxidation-reduction reactions using oxidation states
Applying these rules to the two previous reactions shows that the oxidation states of O and H do not change from their usual values (−2, +1, respectively) in either case. In the first reaction, tin is oxidized (its oxidation state is +2 on the reactant side, and +4 on the product side, by rule 2), while the oxidation state of N in the nitrate anion is +5, and in nitrogen monoxide it is +2 (by rules 3 and 4), so nitrogen undergoes reduction. In the second reaction, chromium is reduced - its oxidation state is +6 in the dichromate (Cr2O72−) anion (by rules 3 and 4) on the reactant side, and is +3 (by rule 2) on the product side. The two carbon atoms in ethanol can be assigned an oxidation state of −2 (it is actually an average in this case - two atoms of the same element can have different oxidation states depending on how they are bonded in the molecule or ion under consideration, but that need not concern us further here), while in the product acetic acid, we arrive at an oxidation state of zero for carbon. We see that the organic compound ethanol is being oxidized to acetic acid by the oxidizing agent dichromate.
Oxidation states of carbon atoms in simple organic compounds
The oxidation levels of carbon atoms in various functional groups will be considered in order to train us to recognize oxidation and reduction in biochemical reactions. The following shows how the oxidation number of the carbon atom changes for the series of one-carbon molecules containing C, H, and O only.

Note the oxidation number for carbon changes in steps of two in concert with the addition or loss of two electrons and as the number of bonds the carbon atom makes to oxygen decreases or increases.
Having assigned oxidation numbers, and understanding that changes in oxidation numbers are the result of adding electrons (reduction) or removing electrons (oxidation), balanced half reactions for oxidations or reductions can be written. For example, the reduction of formaldehyde to methanol shown above can first be written as an unbalanced reduction half reaction,
CH2O + 2e− → CH3OH
Since the equation is unbalanced with respect to charge and hydrogen atoms, acidic conditions are typically assumed in cases such as this (meaning that we add H+ on either side of the equation as required to balance both hydrogen atoms and charge), and the balanced reduction half reaction can be written:
CH2O + 2H+ + 2e− → CH3OH
Reactive oxygen species
A similar series for the simplest oxygen compounds is of biological importance. The energy-yielding process of oxidative phosphorylation results in the reduction of molecular oxygen to water. Both oxygen and water are themselves benign, such is not true for intermediates that are only partially reduced to water. Although the molecular choreography of oxygen reduction in cells that carry out oxidative metabolism is tightly and precisely channeled so as to minimize the possibility of their release, occasionally and inevitably a partially reduced oxygen species is produced.
"...danger lurks in the reduction of O2."
This dire warning appears in a popular biochemistry text (Ref. 4), and it refers to these partially reduced oxygen intermediates, which are collectively termed reactive oxygen species (ROS). Because of their extremely reactive nature, ROS are harmful to cells, chemically damaging membrane lipids, proteins, and nucleic acids. Let's summarize these species and their oxidation states, which are intermediate between molecular oxygen and water (the oxidation numbers shown are for the oxygen atom[s]):
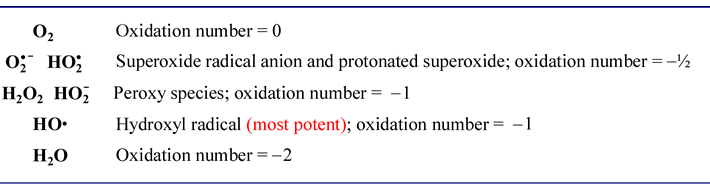
Redox chemistry and electricity
Charge (q) is measured in Coulombs (C)
Charge of an electron: 1.60217653 × 10−19 C
The Faraday (F), the charge of 1 mol elementary charge:
F = 9.648534 × 104 C · mol−1 = 96.485 kJ ·V−1· mol−1
Current (I) is measured in amperes (A). An ampere is defined as 1 A = 1 C · s −1
Voltage and electrical work. The flow of water is a useful analogy for electric current. The flow as measured by volume of water per unit time would be like the flow of charge per unit time. Furthermore, the gravitational potential difference (in height of a column or reservoir of water above the level where flow is measured) is analogous to the electric potential difference existing between two charge "reservoirs". When charge flows "downhill" through an electric potential gradient, it can do useful work, just as a mass of water flowing downhill toward lower gravitational potential can do the work of turning a turbine (and thereby generating ....electric potential energy!). Electric potential is measured in volts, where a volt is defined as 1 V = 1 J · C −1 . In other words, a charge of 1 C moving through a potential difference of 1 V is equivalent to 1 J of work (work is equivalent to energy, as shown by the work-energy theorem of physics). Note that 1 J · V −1 = 1 C is a convenient conversion factor for charge. (This is used above in the unit conversion for the Faraday constant.) For reasons of clarity, the potential difference quantity, also referred to as electromotive force (emf), will be represented here as ΔE.
Redox processes and electron carrier molecules in biochemistry
A great many biochemical reactions are oxidation-reduction reactions, so in a sense the participants in these reactions that undergo oxidation or reduction are electron carriers. In order for redox processes to serve as a source of energy in the form of an emf through which electrons flow, organisms utilize (heterotrophs) or are able to create (autotrophs) molecules that are reducing agents. These electrons get passed from one electron carrier to the next, in a series of electron transfer reactions. Electrons flow exergonically from species with a lower to a higher reduction potential. Reduction of a terminal electron acceptor, such as O2 in aerobic metabolism yields an end product which is exchanged with surroundings.
There are recurring patterns of redox reactions in biochemistry. Metabolic intermediates that are relatively reduced, derived for example from the the food consumed by heterotrophic organisms, such as glucose 6-phosphate or pyruvate, can be oxidized with electrons being transferred to the oxidized forms of redox cofactors that serve as modular cosubstrates in enzyme-catalyzed redox reactions. The enzymes involved are in the oxidoreductase class of enzymes, and many of these work with one of two modular cosubstrates, nicotinamide adenine dinucleotide and flavin adenine dinucleotide.
Nicotinamide adenine dinucleotide (NAD) is an important, ubiquitous redox cofactor
that functions as a carrier of electron pairs. The oxidized form of the cofactor carries
a positive charge, and is denoted NAD+ while the reduced
form is NADH. The nicotinamide portion of NAD+,
consisting of a carbamylated pyridine ring (in red in the figure below,
corresponding to niacin, one of the B-complex vitamins
acts as the electron pair acceptor.
In undergoing reduction, the 4-position of the NAD+ ring
(para to the nitrogen atom) in effect accepts a hydride ion (H:−).
Note that the "dinucleotide" part of the name is due to the fact that the other
"half" of NAD is an adenine-containing nucleotide, AMP.
The two "halves" of the molecule are linked by a phosphoanhydride bond.
A closely related cofactor, NADP+, (reduced form, NADPH) differs only in having a phosphate attached to the 2′ position of the ribose attached to adenine. NADPH generally functions in redox reactions in biosynthetic pathways (e.g. fatty acid synthesis, whereas NAD+ predominates in catabolic processes, such as those associated with glycolysis and oxidative phosphorylation.
The NAD+/NADH redox half-reaction (see figure below) has a standard biochemical reduction potential, ΔE°′ of −0.315 V. The progress of reactions involving NAD+/NADH can be conveniently monitored spectrophotometrically due to the appearance of a broad absorption with its peak at 340 nm when NADH is formed.
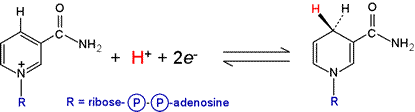
The major source of NADH in oxidative metabolism is the citric acid cycle. The NADH produced is reoxidized to NAD+ when the former donates its electrons to the first component of the electron transport chain (ETC). Eventually these electrons reduce molecular oxygen to water.
Flavin adenine dinucleotide (FAD)
is an important, ubiquitous redox cofactor, consisting of (like NAD)
an AMP
moiety in an anhydride linkage to FMN (flavin mononucleotide,
shown in blue in the figure). The isoalloxazine ring system of
FAD or FMN can accept one or two electrons, in contrast to NAD,
which can only be reduced by two electrons at a time. In the
electron transport chain (ETC)
FMN acts as a cofactor in the NADH-Q oxidoreductase
complex, accepting 2 electrons from NADH, and transferring them to a series
of iron-sulfur (Fe-S) proteins,
and then to coenzyme Q (ubiquinone).
Electrons from FADH2 enter the electron transport chain at the
level of cytochrome reductase, the second proton-pumping complex in the
electron transport chain (downstream from NADH-Q reductase),
via the succinate-Q oxidoreductase complex,
which accomplishes the transfer of electrons from FADH2 to coenzyme Q
(again through Fe-S proteins).
The full structure of FAD is shown below.
The three rings at the top constitute the isoalloxazine ring system,
or flavin portion of the molecule.
The portion of the molecule corresponding to FMN (shown in blue) also includes the residue
of the five-carbon D-ribitol
(a polyhydroxy alcohol derived from the sugar D-ribose
with an attached phosphate group.
The isoalloxazine ring plus ribitol corresponds to riboflavin,
one of the B-complex vitamins.
FMN is linked to an adenosine monophosphate (AMP) shown in black) by a phosphodiester bond. The reduction of FAD involves the 1 and 5 nitrogen atoms (labelled red in the figure at left), and the oxidation states of FAD/FMN are shown in the figure below. The molecule labelled (1) represents FAD or FMN - the most oxidized form. The molecule labelled (2) is a radical or semiquinone formed by a one electron reduction of (1). A second one-electron reduction converts the radical to (3), which represents the fully reduced forms FADH2 or FMH2.

Related topics pages:
- electron transport chain (ETC)
- Problems related to biological oxidation-reduction reactions